Part 1 of this two-part report described the beginnings of radiation oncology in the United States, including many of the field’s early pioneers and the rise of associated professional societies. In part 2, we will consider the advances in technology and biology that are the foundation of modern radiation oncology.
Early Technology
The rudimentary tubes initially used to produce x-rays had limitations in terms of the electrical current and penetrating power to kill cancer cells in deep-seated tumors. By evacuating the air from the tube (“vacuum” tube) and adding a heated grid between the anode and the cathode, the production of electrons that could be repelled from the cathode and accelerated toward the anode was increased, thus increasing the yield of x-rays to treat the cancer and decreasing the duration of each treatment. But the depth of penetration was dependent upon the energy of the photons, and that was dependent upon the electrical potential difference that could be applied between the two electrodes.
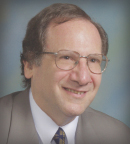
Christopher Rose, MD, FASTRO
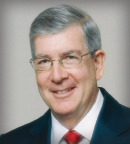
Allen S. Lichter, MD, FASTRO, FASCO
By the 1920s, reliable x-ray tubes were being produced in Europe and the United States, with energies of 200,000 electron-volts (200 keV orthovoltage). They allowed for the treatment of tumors within 2 cm of the skin surface, but the maximum dose still resided on the skin surface, causing erythema and ulceration. For deeper-situated tumors, the surgeons of the early part of the 20th century turned to Marie and Pierre Curie, who had discovered naturally occurring higher-energy x-radiation from uranium and radium. Mme. Curie’s second Nobel Prize in Chemistry recognized her work purifying radium from pitchblende and providing sources of pure radium and radon gas to cancer centers in Europe and the United States.
Since the isolation of radium was such a laborious procedure, only a few cancer centers had access to enough of it to allow for external irradiation of the deep-seated tumors with so-called radium bombs (Figure 1).1 The pre–World War II (WWII) radiation treatment centers primarily used vacuum tubes, and technical advances allowed for increasing energy but still to less than 1 million electron-volts.
“As the technology for generating high-energy x-rays advanced, so too did the science of the biological effects of radiation, or radiobiology.”— Christopher Rose, MD, FASTRO, and Allen S. Lichter, MD, FASTRO, FASCO
Tweet this quote
In the immediate post-WWII era, various methods to increase electron energy using electrons accelerated around oval-shaped magnet-restrained accelerators (beta particle cyclotrons, or bevatrons), rapidly moving conveyor belts containing electrons delivered to a tungsten target (Van de Graaff generators), and resonating microwave transformers. These devices created the first nonisotope source of supervoltage x-rays (> 1 MeV) that deposited their peak energy beneath the subcutaneous tissues and were able to treat deeper-seated tumors.
Linear Accelerators
The problem with these technologies was that the machines were huge and unwieldy to aim (Figure 2).1 Radar technology, developed during WWII, allowed for the creation of compact linear electron accelerators (linacs), small enough to be placed into an orthovoltage room with a rotating gantry, which allowed for irradiation along a 360-degree plane around the patient. The first linear accelerator was installed in a hospital in London in 1953. The first linac in the United States was developed at Stanford University in 1956 as a collaboration between Varian Associates and Henry Kaplan, MD, Professor of Radiology at Stanford.2
Early linac technology was sufficiently unreliable that only a few large academic hospital-based medical centers could afford the on-site service engineers and infrastructure. As an alternative, physicists and engineers developed cobalt-59 sources that were placed in nuclear reactors, and the artificial nuclide cobalt-60 was produced by neutron activation. Cobalt-60 decayed with the release of two supervoltage gamma rays with a mean energy of 1.25 MeV. Cobalt-60 sources were sufficiently small and could be shielded by lead, fit within a gantry in a small orthovoltage room, and collimated to treat deep-seated tumors and avoid normal tissue adjacent to the tumor. By the late 1950s, most community and academic cancer centers in the United States had acquired cobalt machines, retiring their large betatrons and relegating their conventional x-ray equipment to treating skin cancer.
Beyond the Linac
By the late 1960s and 1970s, linear accelerator technology had become sufficiently reliable that community hospitals and academic medical centers could swap out cobalt and obtain the benefits of increasing photon energies and multiple electron energies to treat superficial and deep superficial tumors with sharper edges and lower doses to normal tissue adjacent to the tumors. Computed tomography (CT) scanners, developed initially by the British company EMI, allowed for cross-sectional diagnostic imaging and, for radiotherapy, more precise tumor localization than could be obtained by planar imaging. At many academic centers in the United States, radiation oncologists and physicists integrated the cross-sectional images and developed in-house software routines to move treatment planning from a single slice to dosimetry along the entire treatment volume.
In Ann Arbor, University of Michigan physicians and their physics colleagues Randall Ten Haken, PhD, Benedict Fraass, PhD, and Dan McShan, PhD, developed one of the first user-friendly three-dimensional (3D) treatment planning systems—known as the UM-Plan—including “beam’s-eye view” visualization. The Michigan group used this system to allow for dose escalation in prostate, lung, and brain tumors and demonstrated the power and utility of 3D treatment delivery to improve tumor outcomes while respecting normal tissue tolerance.3
Computer control of dose-rate, gantry and table position, and collimator shape was incorporated into commercial systems in the 1990s and was a prerequisite for the subsequent development of intensity-modulated radiation therapy (IMRT). Now routine for most curative radiation, IMRT allows for “dose painting”—the ability to deliver differential doses to tumor volumes and specify regions adjacent to the tumor, where higher doses are steered away.
Radiobiology
As the technology for generating high-energy x-rays advanced, so too did the science of the biological effects of radiation, or radiobiology. The field was founded on:
- The development of techniques to culture individual cells to assess radiation damage
- An understanding of cell-cycle kinetics
- The concept of a continuum from tissue and tumor stem cells to fully differentiated cells
- An awareness of how ionizing radiation damaged DNA directly and indirectly
- These concepts led to the so-called 4Rs of radiobiology: repair, reassortment, reoxygenation, and repopulation.
In 1959, Mortimer Elkind, PhD (Colorado State University) described the ability of cells not killed by radiation to repair damage so that by 24 hours later, the previously irradiated cell demonstrated the same radiation tolerance without any seeming effect of the prior radiation.4
It was then demonstrated that photon radiation interacted with the cellular water creating free radicals (hydronium ions and aqueated electrons) that interacted with DNA, causing DNA damage, mostly single-strand breaks. Oxygen was key to stabilizing these free radicals. However, in a hypoxic state, these free radicals would more easily recombine, thus reducing the amount of DNA damage. Tumors had regions of hypoxia associated with decreased radiosensitivity; immediately after radiation, oxic tumor cells were killed more than the hypoxic cells by a factor of three. Fractionation of radiation doses allowed for tumor shrinkage and reoxygenation of the previously spared hypoxic cells. In addition, attempts were made to develop drugs that would overcome the effects of hypoxia, the so-called oximimetic hypoxic cell sensitizers (eg, misonidazole, tirapazamine).
Hyper- and Hypofractionation
If once-daily fractionation of the radiation dose was advantageous, possibly multiple factions per day would be even better. By the 1990s, several human trials had evaluated hyperfractionation, with 7,440 cGy in 1.2-Gy fractions twice a day as the most prevalent regimen. (The gray, or Gy, is the international unit defined as the absorption of 1 joule of energy per kilogram of matter.) Some investigators pushed the paradigm to a practical limit, delivering 54 Gy in 36 1.5-Gy fractions three times a day over 12 consecutive days. Although normal tissue tolerance was maintained and local control was improved over conventional daily fractions of 1.8 to 2 Gy per day, cure rates were not improved, with patients dying of systemic tumor progression.
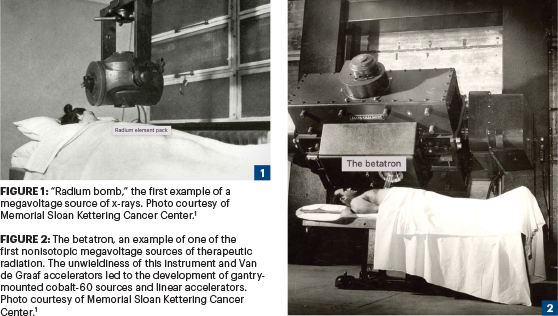
In the early 1990s, radiation oncologists and neurosurgeons began to employ even more highly conformal technologies (proton beam at Massachusetts General Hospital [MGH] and the University of California, San Francisco [UCSF]; Gamma Knife at the University of Pittsburgh Medical Center) to treat brain metastases and functional lesions with single fractions. This ultimate hypofractionation regimen stood in stark contrast to the decades-old paradigm of many small daily doses strung together in sequence.
Wendell Lutz, PhD, Ken Winston, MD, and Jay Loeffler, MD, at Harvard modified a linac using a special head frame, collimator cones, and noncoplanar dose delivery to create treatments and dosing patterns like the Gamma Knife, but with the standard linac used by radiation oncologists. David Larson, MD, PhD, took the technology to UCSF, where he joined the Brain Tumor Cooperative Group headed by Charles Wilson, MD, and Glen Sheline, MD. He intuited that most radiosurgery targets had no normal tissue within them. So, the concern to spare normal tissue by fractionation was irrelevant and subsidiary to controlling the tumor. Patients with brain metastases achieved high rates of local control, and patients with benign indications (eg, arteriovenous malformation and benign pituitary tumors) were also controlled, with minimal toxicity to the adjacent normal tissue.5
Could large-dose hypofractionation have a role in non–central nervous system sites? Unlike targets in the brain, tumors in the body are plastic: they move and change shape as a function of respiration, cardiac movement, peristalsis, and body movement. Conventional radiation was tolerant of these movements and shape changes due to the large margins placed around the visible tumor.
Robert Timmerman, MD, working initially at Indiana University School of Medicine and later at The University of Texas Southwestern, extended this ablative approach to stages I and II non–small cell lung cancer in frail patients who could not undergo surgery. Using a body mold and limiting diaphragmatic motion, he demonstrated high degrees of control with minimal toxicity. Advances in treatment delivery and motion management have allowed for the extension of this method, generically called stereotactic body radiation therapy (SBRT) or stereotactic ablative body radiation (SABR), to treat most of the common body sites including breast and prostate, and particularly extending the utility of radiation to tumors that were labeled “radioresistant” such as kidney and adrenal cancers, sarcomas, and pancreatic cancer.6 The addition of CT and even magnetic resonance imaging (MRI) into linear accelerators has facilitated this precision treatment, even allowing one to view the target tissue during treatment delivery and interrupt the treatment should the target move outside the confines of the beam.
We have now come full circle. In the 1920s, high doses given in a single or few sessions could eradicate the tumor but at the cost of normal tissue necrosis. This problem was addressed by fractionating the dose into 20 to 30 smaller treatments given daily over 4 to 6 weeks. This approach was taken to the extreme with a hyperfractionated approach, delivering treatments twice or even three times a day. But now—with the ability to manage motion and tightly conform and control the dose distribution—we are moving back toward a small number of high-dose treatments delivered over a short period.
Protons
Photons and electrons lose their energy exponentially as they enter the body. Normal tissues anterior to the tumor will receive higher doses than the tumor, and, thus, cross-firing fields are necessary to achieve therapeutic gain. Protons offer a means of depositing the maximum energy within the designated volume from each single port, thereby minimizing normal tissue effects.
Physicist Robert Wilson, PhD, working at the Lawrence Berkley Lab, proposed using protons for cancer treatment in 1946. By 1954, protons were first used by John Lawrence, MD, to treat small pituitary targets at the Lawrence Berkley Lab. A cyclotron at the Harvard Cyclotron Lab was used for similar targets by neurosurgeon Raymond Kjellberg, MD, in 1963. With the arrival of Herman Suit, MD, MSc, PhD, at MGH in 1974, large-field proton radiation began. On both East and West Coasts, the development of this type of radiotherapy was slowed due to problems with tumor localization and inflexibility for medical indications because research accelerators were not designed for treating patients.
In 1984, James Slater, MD, and John Archambeau, MD, began discussions with Dr. Suit, Joseph Castro, MD, at UCSF, and physicists at U.S. national accelerator labs, culminating in the formation of the Proton Therapy Cooperative Group. The group formed three subcommittees to investigate accelerator design, facility design, and clinical questions. In 1986, Dr. Slater approached the director of the Fermi National Accelerator Lab and its university consortium to develop a medical proton accelerator at Loma Linda University.7
In October 1990, the first patient was treated at Loma Linda. The facility has been in continuous operation since then. The MGH team, under the guidance of Dr. Suit and Michael Goitein, PhD, continued to treat patients at the Harvard Cyclotron Lab until the opening of their own proton therapy center in 2001. Commercial proton accelerators have been sited at 37 additional centers across the United States, with 2 more currently under construction.
Oligometastases
Samuel Hellman, MD (of the University of Chicago) observed in the 1990s that there was a spectrum of both the numbers of clinically apparent breast cancer metastases and the rate of disease progression after their recognition. University of Chicago colleague Ralph Weichselbaum, MD, and Dr. Hellman hypothesized that the management of such disease by ablative methods might improve care. They initially used the term “oligometastases” to identify a population with limited numbers of metastases at presentation.8 Their group performed the first nonsurgical clinical trial of SBRT for patients with one to five metastases remaining after successful systemic management for lung cancer and demonstrated ~20% long-term survival in patients without other therapeutic options. Numerous confirmatory phase II studies have been conducted.
“The first 100 years of U.S. radiotherapy have seen numerous discoveries and developments, and the years ahead will see even more.”— Christopher Rose, MD, FASTRO, and Allen S. Lichter, MD, FASTRO, FASCO
Tweet this quote
The European Society for Radiation Oncology (ESTRO) and the European Organisation for Research and Treatment of Cancer (EORTC) have developed a framework for the definition and characterization of both oligometastatic disease and the newer characterized entity, oligoprogressive cancer, which was published in 2020.9 This framework will allow for more precise stratification of patients, more confidence in determining which patients might benefit from local ablative interventions, and an understanding of the mechanism(s) that provide for such benefit.
Looking Toward the Future
The history of radiation oncology in the United States is rich with technological and radiobiological advances over the past century. Outstanding pioneers emigrated from Europe, joining forces with general radiologists who dropped their diagnostic work to focus entirely on therapeutic radiology. In many respects, these practitioners were some of the earliest “oncologists” and established the foundations of the specialty known today as radiation oncology.
We are not done improving our understanding of the use of radiation to treat cancer nor with the technological development of increasingly sophisticated dose delivery equipment and techniques. Radiation can enhance the effects of immuno-oncology agents, and combinations of radiation and chemotherapy drugs continue to form the basis for many curative treatments.
More exotic particles beyond protons have been utilized to treat malignancies (eg, pi mesons, carbon ions) and are certainly going to be studied further. Radiation doses delivered in seconds or portions of seconds (flash radiation) have biological properties that are unique and will find their place in cancer treatment as well. Integration of imaging equipment (CT, MRI, and positron-emission tomography) into the radiation delivery machine is allowing even more precise targeting and dose delivery.
The first 100 years of U.S. radiotherapy have seen numerous discoveries and developments, and the years ahead will see even more.
Acknowledgment: The authors would like to thank Dr. Frank Wilson, Dr. Sam Hellman, and Ms. Beth Bukata of ASTRO for their assistance in reviewing this manuscript.
DISCLOSURE: Dr. Rose is a consultant to CTSI, a Varian company, which is a subsidiary of Siemens Healthineers. Dr. Lichter is a consultant to Ascentage Pharmaceuticals and serves on the Board of Cellworks.
REFERENCES
1. Memorial Sloan Kettering Cancer Center: MSK Radiation Therapy: Timeline of Progress. Available at https://www.mskcc.org/timeline/msk-radiation-therapy-timeline-progress. Accessed April 20, 2022.
2. Baker M: Medical linear accelerator celebrates 50 years of treating cancer. Stanford News, April 18, 2007. Available at https://news.stanford.edu/news/2007/april18/med-accelerator-041807.html. Accessed April 20, 2022.
3. Fraass BA: The development of conformal radiation therapy. Med Phys 22:1911-1921, 1995.
4. Elkind MM, Sutton H: X-ray damage and recovery in mammalian cells in culture. Nature (London) 184:1293, 1959.
5. Phillips T, Sahgal A: An interview with David Larson, MD, PhD, FASTRO. July 1, 2016. Available at https://www.astro.org/About-ASTRO/History/David-Larson, 2016. Accessed April 20, 2022.
6. Timmerman R: A story about hypofractionation and the table on the wall. Int J Radiat Oncol Biol Phys 122:4-21, 2022.
7. Slater JM, Archambeau JO, Miller DM, et al: The proton treatment center at Loma Linda University Medical Center: Rationale for and description of its development. Int J Radiat Oncol Biol Phys 22:383-389, 1992.
8. Hellman S, Weichselbaum R: Oligometastases. J Clin Oncol 13:8-10, 1995.
9. Gluckenberger M, Lievens J, Bourma AB, et al: Characterisation and classification of oligometastatic disease: A European Society for Radiotherapy and Oncology and European Organisation for Research and Treatment of Cancer consensus recommendation. Lancet Oncol 21:e18-e28, 2020.
Dr. Rose served as Associate and Technical Director for Valley Radiotherapy Medical Group Inc from 1983 to 2016; Chief Technology Officer of Vantage Oncology from 2002 to 2016; and Chief Radiation Oncologist of the US Oncology Network, Pharmaceutical Solutions and Services, McKesson from 2016 to 2020. Dr. Lichter served as Chair and Professor of Radiation Oncology at the University of Michigan from 1984 to 1998; Dean of the University of Michigan Medical School from 1998 to 2006; and Chief Executive Officer of ASCO and Conquer Cancer: the ASCO Foundation, from 2006 to 2016.